Water fasting and dry fasting. The human body has an amazing ability to adjust to not having food or water for a long time, which was very important for early humans to survive. Can you imagine our ancestors dying after not finding a source of water for a few days?
Since we now know the mechanisms of pure dry fasting, we now know that it is preferable in the short term specifically for muscle preservation as opposed to low caloric eating.
Understanding these changes can help us better understand certain medical conditions involving ketosis, what to expect, and how to use it to our advantage in both a healing and longevity sense.
Let's quickly summarize our understanding of starvation and ketosis. When the body goes without food for a long time, it tries to save important nutrients like nitrogen. The brain starts to use more keto acids, which means it doesn't need as much glucose. This change in fuel use helps the body keep more amino acids in the muscles instead of turning them into glucose.
Initially, the body eliminates nitrogen via urine in the form of urea. As time progresses, it shifts to using ammonia, which assists the kidney in utilizing more glutamine. It's also a main mechanism of buffering your blood glucose during a fast. Amonnia mixes with ketoacid salts and produces bicarbonate. The kidney then converts glutamine into glucose and generates more HCO3−.
As the body transitions from expelling nitrogen as urea to ammonia, it supports urine flow and waste removal. Over time, nitrogen loss in urine decreases as the kidneys become more efficient at absorbing ketone bodies. The body also adjusts sodium levels in urine to maintain stable potassium levels and prevent the formation of uric acid crystals.
It is worth mentioning that males and females may exhibit distinct responses to starvation.
Ammonia and Acid from breaking down proteins
Our bodies produce acid daily as a result of breaking down proteins. To maintain a healthy acid-base balance, the kidneys create and excrete substances, such as ammonia. The kidneys use an amino acid called glutamine to create ammonia and bicarbonate. Bicarbonate is then absorbed back into the bloodstream to help buffer acids, while ammonia can either be removed through urine or return to the bloodstream.
If ammonia is removed through urine, it results in the formation of new bicarbonate to help maintain the acid-base balance. If it returns to the bloodstream, the liver processes it and consumes an equal amount of bicarbonate, essentially neutralizing the bicarbonate produced during kidney ammonia production.
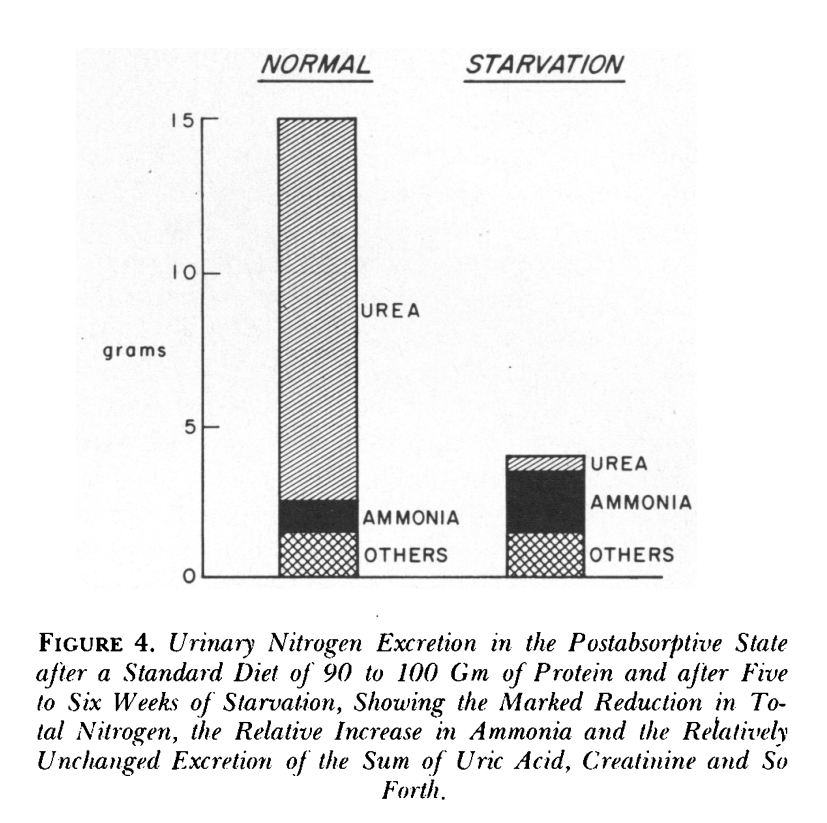
The kidneys play a critical role in regulating acid-base homeostasis by controlling ammonia production, movement, and excretion. In response to changes in the body's acidity, such as during metabolic acidosis or alkalosis, the kidneys adjust how much ammonia they excrete through urine.
This process involves different parts of the kidneys, such as the proximal tubule, thick ascending limb of the loop of Henle, and the collecting duct, working together to selectively transport ammonia into either the urine or the bloodstream.
In various clinical conditions, the changes in urinary ammonia excretion become the primary mechanism by which the kidneys regulate acid excretion. This integrated kidney function ensures the maintenance of a healthy acid-base balance in the body, even under different metabolic circumstances.
Postabsorptive Phase
During the initial day of fasting, the absence of dietary glucose causes blood sugar levels to drop, leading to reduced insulin and increased glucagon levels.
Glucagon helps the liver release glucose from its glycogen stores, and the drop in insulin minimizes glucose transport to muscles and fat cells, ensuring the brain and glycolysis-dependent tissues, like red blood cells, testicles, kidney medulla, and bone marrow, have enough glucose.
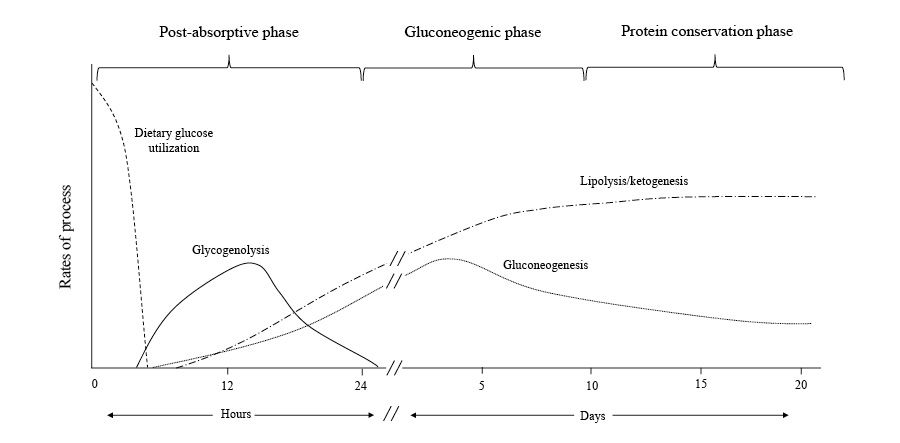
In this stage, liver glycogenolysis supplies around 75% of the body's glucose needs. The liver's glucose-6-phosphatase enzyme converts glucose-6 phosphate into free glucose for other cells. Although muscle glycogen contributes as well, it is first converted to lactate, which is then transformed back to glucose in the liver through the Cori cycle.
Gluconeogenesis, using lactate, pyruvate, and glycerol as building blocks, accounts for approximately 10-15% of the remaining glucose requirement. Although the Cori cycle doesn't increase net glucose levels, it helps to limit protein breakdown in the early stages of fasting.
Where does lactate and glycerol come from when the body is searching for glucose to satisfy the glycolytic cells (cells that can only function on glucose)? Adipocytes, commonly known as FAT, that's where. A lot of people are unaware that both protein and fat can give our body glucose, and are the main drivers of gluconeogenesis and the ability to fast for extended periods of time. Adipocytes convert to lactate and glycerol while protein converts to lactate and pyruvate. Both of these need to go through the liver to turn back into glucose. The beautiful thing about the liver is that it is our bodies best store of glycogen, which it easily turns into glucose. The secondary glucose channels require a little bit more work, and therefore it's natural to feel more sluggish and have a longer recovery time when in a fasted state. Have you ever tried to run a short distance while a few days into your fast? You'll notice you feel great for a short burst of time and then crash. This is because getting glucose back into your muscle cells is taking a lot more time and you're putting a big strain on your body, which is trying to prioritize the glycolytic cells. Cells like the kidney, certain parts of the brain, and bone marrow so that it can fuel the red blood cells. Your muscles come last.
With reduced insulin, lipolysis is triggered, making fatty acids an alternative energy source for muscles in this phase's later stages. The oxidation of fatty acids produces acetyl CoA, which inhibits pyruvate dehydrogenase.
This process ensures that the limited glucose absorbed by muscles is preferentially converted to pyruvate and lactate, which are then transformed back to glucose in the liver. The liver uses fatty acid mobilization and oxidation to generate energy for glucose production, preserving protein by decreasing the demand for amino acids in gluconeogenesis.
Gluconeogenic Phase
After fasting for 24 hours, when glycogen reserves run out, the body transitions into the gluconeogenic phase. In this stage, the brain relies on glucose produced from amino acids, lactate, pyruvate, and glycerol.
- Gluco: Meaning glucose.
- Neo: Meaning new.
- Genesis: Meaning origin or creation.
Lactate: Lactate or lactic acid is the major gluconeogenic substrate. It’s derived from pyruvate — the direct product of glucose or glycogen breakdown. When you do an intense workout, your cells eventually turn pyruvate into lactate because it can be used for energy, and lactate accumulates in your muscles. What most people don’t know is lactate can be turned into pyruvate once again and back into glucose — aka gluconeogenesis.
Glucogenic amino acids (aka protein): Amino acids can be divided into ketogenic (stimulate ketone production), glucogenic (stimulate glucose production), or both. Every single amino acid can be turned into glucose except for lysine and leucine, which are exclusively ketogenic. The main amino acids used for gluconeogenesis are alanine and glutamine. On average, you need 1.6 g of amino acids to make 1 g of glucose, which is expensive.
Glycerol: After lactate and glutamine, glycerol is the third most used substrate. It comes from fat breakdown.
Persistent low insulin levels lead to muscle proteolysis, supplying the necessary materials for increased liver gluconeogenesis. During this period, alanine and glutamine are the primary amino acids released from skeletal muscles.
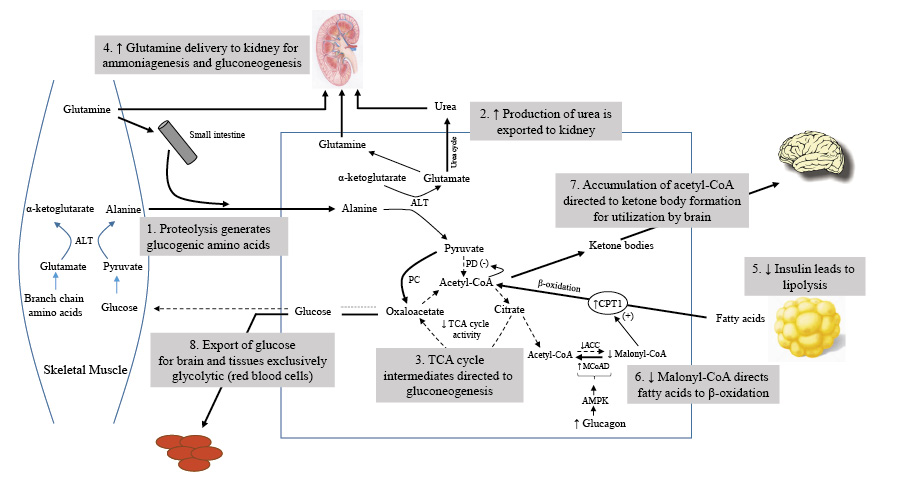
The body employs branched-chain amino acids (BCAAs) to create alanine, which is then transformed into glucose by the liver, while its amino group becomes urea and is excreted through urine. This mechanism helps control gluconeogenesis without raising blood ammonia levels. Moreover, insulin can suppress the liver's uptake of alanine, further regulating gluconeogenesis.
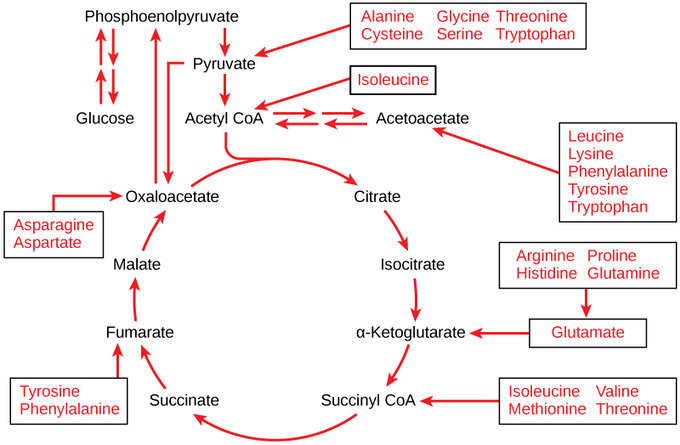
Glutamine, derived from other amino acids, serves as a crucial energy source for cells in the intestinal mucosa and the immune system. It is also the main substrate for kidney gluconeogenesis, where ammonia production plays a key role in maintaining acid-base balance.
As the gluconeogenic phase progresses, fatty acids are increasingly directed to the liver for ketone body production. The accumulation of acetyl CoA ensures that pyruvate is utilized for gluconeogenesis by inhibiting pyruvate dehydrogenase.
The generation of keto acids enables the liver to manage the large amounts of acetyl CoA produced, while the brain's growing consumption of ketone bodies helps conserve glucose for tissues that depend on glycolysis, such as red blood cells, bone marrow, and the kidney medulla.
Conservation of Protein Phase
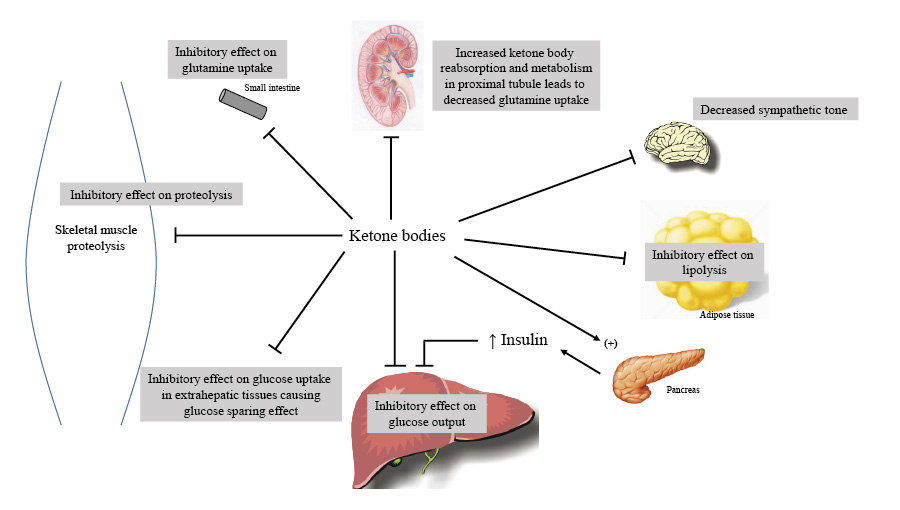
The brain's glucose consumption decreases from 120 grams per day during the initial 24 hours of food deprivation to around 40 grams per day after several weeks of fasting. The breakdown of muscle protein declines, mainly due to decreased release of muscle alanine, as ketone bodies help inhibit muscle proteolysis and adapt to extended fasting.
Lactate, pyruvate, and glycerol, released during lipolysis, contribute to the liver's remaining glucose production. Glucose is utilized in red blood cells, bone marrow, and kidney medulla, which rely exclusively on glucose for energy through glycolysis.
An increase in kidney gluconeogenesis occurs alongside a reduction in hepatic gluconeogenesis. The kidney extracts more glutamine, producing ammonia for the urinary excretion of ketoacid salts and glucose synthesis. This process generates new bicarbonate to counterbalance the consumption of bicarbonate in ketoacid buffering. As muscle protein breakdown decreases, ammonia replaces urea as the primary nitrogenous waste product in urine, preventing oliguria.
Eventually, ketone bodies reach equilibrium, resulting in starvation ketosis, characterized by a plasma bicarbonate concentration of approximately 18 mEq/L and a β-hydroxybutyrate concentration of 8-10 mmol/L. Ketone bodies significantly contribute to this new balance by promoting insulin release and suppressing lipolysis in fat cells.
Keto acids and fatty acids gradually supplant glucose as the preferred energy source for skeletal and cardiac muscles. Ultimately, the utilization of free fatty acids becomes dominant, reserving keto acids for brain function. This state of reduction is associated with a decline in muscle proteolysis, enhancing the nitrogen-conserving effects of keto acids in skeletal muscle.
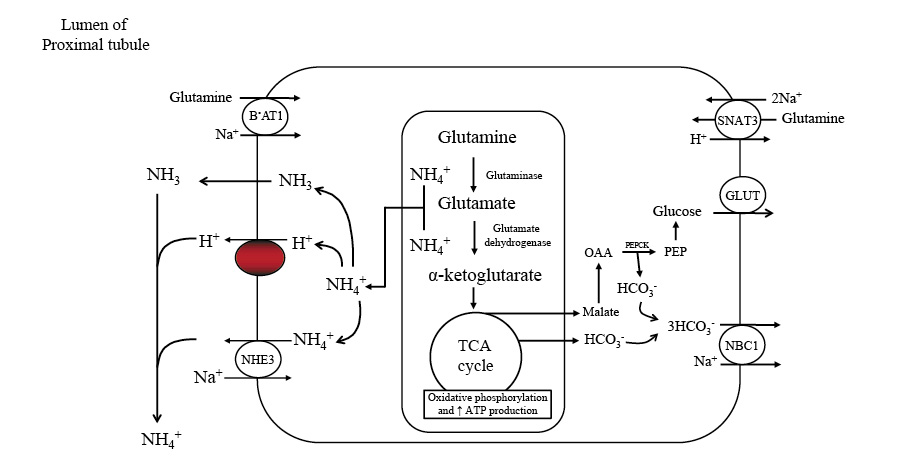
Sources



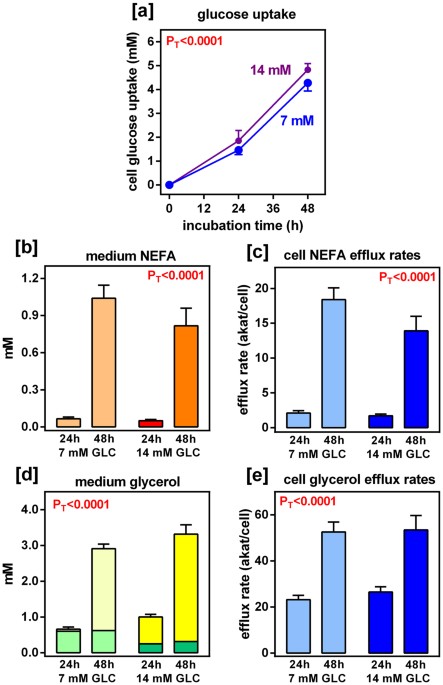